Quantum mechanics is often counterintuitive and difficult to comprehend. The behavior of matter on a small scale is so fundamentally different from anything on a large scale, that we cannot paint a sharp picture of an atom or an electron which correctly explains how it is going to behave. We need the mathematical expressions of quantum mechanics to calculate what will happen.
In quantum electronics we aim to understand, control and manipulate spin states and topological states in nanoscale devices. In our experiments we perform energy- and magnetic spectroscopy in electron transport measurements at low temperatures.
In our quantum electronics team we investigate
(i) Spins confined to quantum dots and single dopants
(ii) Topological properties in super-semi hybrid nanowire devices.
We use these material systems:
• Single donor and acceptor atoms in silicon
• Silicon MOS quantum dots
• Cubic and hexagonal germanium nanowires
• Topological PbSnTe and Ge/Si nanowire devices
Silicon Quantum Electronics studies silicon hardware platforms as fundamental building blocks for quantum applications, including quantum computers, quantum sensors and quantum simulators. It relies on controlling the quantum state of electrons or holes confined in Si nanostructures. Specifically, we study the physical limitations of the spin lifetimes, such as the spin-orbit and hyperfine interactions. We employ two different approaches to isolate electrons and holes in precisely-engineered Si nanostructures. We explore p-type and n-type Si MOS QD devices fabricated in the MESA+ Nanolab, the cleanroom facilities at the UT, next to similar devices fabricated by industrial collaborators at IMEC using a 300mm process.
Single donor and acceptor atoms in silicon When a group-V donor or a group-III acceptor atom replaces a Si atom in the lattice, a confinement potential is created. As a result, the donor electron (acceptor hole) remains bound to the nucleus at low temperatures. The spin of the donor electron (acceptor hole) and the nucleus then forms a well-defined quantum system, which can be used to encode spin qubits.
At NE, we design, fabricate and characterize single-dopant atoms in Si, to realize highly-coherent spin qubits, currently focusing on Bi-implanted silicon devices. We aim to realize the first single-atom bismuth (Bi) spin qubit () and to address the qubit at clock transitions, where extended coherence times have been theoretically predicted and experimentally observed for Bi clusters [1].
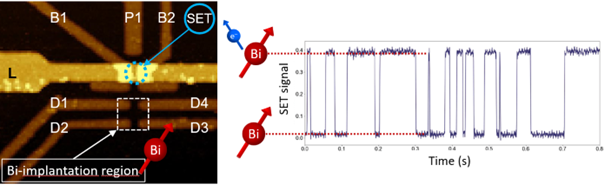
Fig 1: Left panel: Atomic force microscope image of a device implanted with bismuth donors, which are sensed by a single-electron transistor (SET). After Bi implantation, we define electrodes by means of electron-beam lithography, metal evaporation and lift-off techniques. Gates L, B1, P1 and B2 control the tunnel barriers, source, drain and the island of the SET. D1-D4 are meant to tune the ionization of the donors.
Right panel: The SET current can sense whether or not an electron is bound to donor atoms. An electron can tunnel from the donor site to and from the SET, resulting in a jump in the SET signal. The line trace shows real-time detection of tunnel events of the donor ionization
Are you interested in designing, fabricating, and measuring quantum dot devices or Bi-implanted silicon devices? For more information, please do not hesitate to contact us:
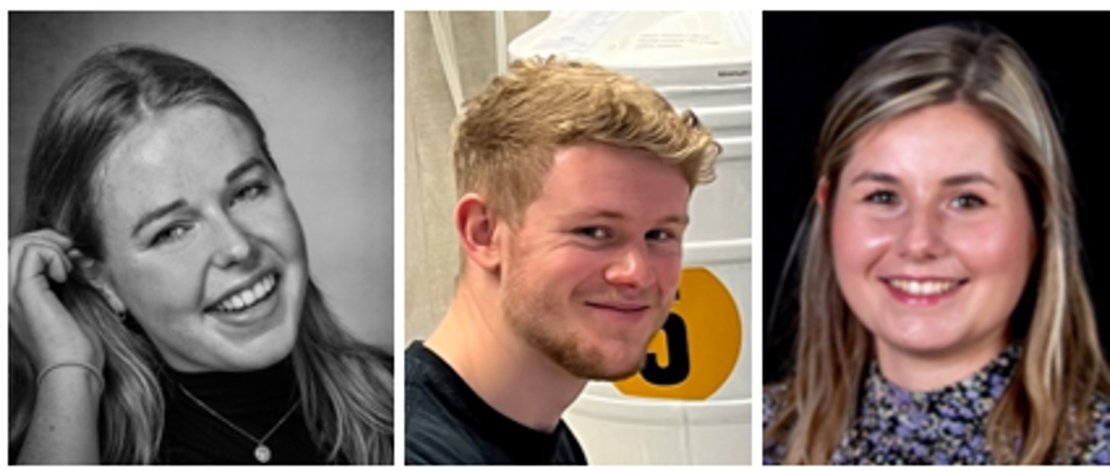
[1] G. Wolfowicz et al., Nature Nanotechnology 8, 561‐564 (2013).
In Si Metal-Oxide-Semiconductor (MOS) quantum dot (QD) systems, the well-established infrastructure of conventional transistors is used to form and control QDs at the Si/SiO2 interface. The QD can be controlled electrostatically using nanoscale gate electrodes, see device picture. The spin state of an electron confined to the silicon QD can be initialized, read out and rotated via single electron spin resonance. Such driven coherent oscillations of an electron spin constitute the realization of spin quantum bits (qubits), the building blocks of a quantum computer.
Spin qubits hosted in Si QD structures have demonstrated excellent performance on the single- and two-qubit level [2]. This, in combination with its small footprint, flexibility in architecture and the possibility to employ the existing semiconductor industry for fabrication, makes spin qubits in electrostatic-defined Si QD platforms suitable for realizing a large-scale quantum processor. Spin qubits hosted by Si MOS QDs have shown long phase coherence and relaxation times [3,4]).
Are you interested in designing, fabricating, and measuring quantum dot devices or Bi-implanted silicon devices? For more information, please do not hesitate to contact us:
[2] A. Laucht, et. al., Roadmap on quantum nanotechnologies. Nanotechnology, 32(16) (2021).
[3] L. Petit et. al., Physical Review Letters, 121(7), 1–8 (2018).
[4] A. Zwerver et. al., Nature Electronics 5, 184–190 (2022).
Cubic and hexagonal Ge/Si nanowires 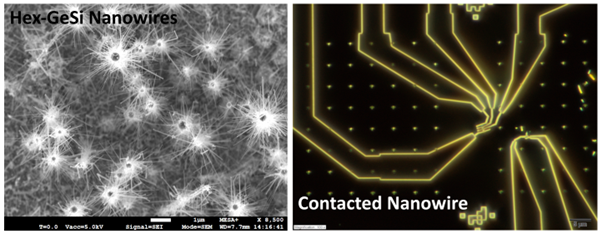
Semiconductor nanowires offer intrinsically strong confinement in two dimensions, making them an ideal platform to realize spin qubit systems. We explore their fundamental properties, such as the spin-orbit coupling strength, g-factor, and spin decoherence mechanisms. Previous experiments on cubic Ge/Si nanowires [3, 4] have already confirmed their potential.
In this project, the nanowires are made from a novel material, called hexagonal Ge/Si [1]. Due to the hexagonal arrangement of the atoms, the nanowire can efficiently emit and absorb photons [2], unlike cubic Ge/Si. This may solve the problem of long range information exchange, e.g. by forming a “photon data bus” for quantum communication.
Are you interested to help realize spin qubits in novel hexagonal Ge/Si nanowires? Do you want to get to know the ins and outs of nanofabrication? For more information, please do not hesitate to contact us:

[1] A. Li et al., Nanotechnology 34, 015601 (2023).
[2] E.M.T. Fadaly et al., Nature 580, 205–209 (2020).
[3] M. Brauns et al., Appl. Phys. Lett. 109, 143113 (2016).
[4] M. Brauns et al., Phys. Rev. B 94, 041411(R) (2016).
Topological PbSnTe and Ge/Si nanowire devices Combining semiconducting materials with a superconductor can lead to exotic physics. Theoretical predictions in 2010 [1] showed for instance that Majorana bound states can form in these hybrid super-semi devices. In our group, we want to understand the physics in these hybrid devices. Furthermore, we look for signatures of topological superconductivity. This effect can be obtained by making use of the superconducting proximity effect in a semiconducting nanowire. Topological superconductivity is a necessity to create a topological quantum computer [2].
Our group uses two types of nanowire materials to investigate signatures of topology: (i) SnTe, an intrinsic topological crystalline insulator (TCI) which should host topological surface and hinge states, and (ii) cubic Ge-Si core-shell nanowires [3] in which the topological states can be engineered by a combination of the semiconducting nanowire properties, superconducting electrodes [4,5] and an external magnetic field.
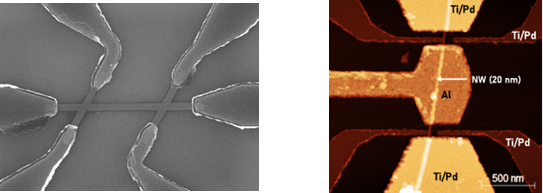
Figure 1: Left: SnTe in-plane nanowire connected with six Ti/Al contacts. Right: Ge-Si core-shell nanowire with a superconducting Al island and Ti/Pd tunnel gates and contacts.
Are you interested in searching for signatures of topological superconductivity in nanowires? Do you want to know what it’s like to work in the MESA+ Cleanroom facilities? Do not hesitate to contact one of us:
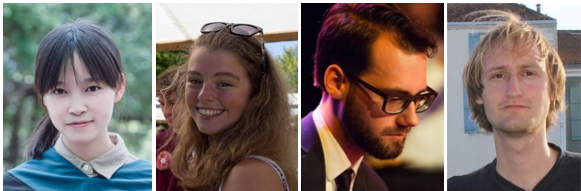
[1] Lutchyn, Roman M., Jay D. Sau, and S. Das Sarma. “Majorana Fermions and a Topological Phase Transition in Semiconductor-Superconductor Heterostructures.” Physical Review Letters 105.7 (2010): 077001.
[2] Kitaev, A. Yu. “Fault-tolerant quantum computation by anyons.” Annals of physics 303.1 (2003): 2-30.
[3] Conesa-Boj, Sonia, et al. “Boosting hole mobility in coherently strained [110]-oriented Ge–Si core–shell nanowires.” Nano letters 17.4 (2017): 2259-2264.
[4] Ridderbos, Joost, et al. “Hard superconducting gap and diffusion-induced superconductors in Ge–Si nanowires.” Nano letters 20.1 (2019): 122-130.
[5] Ridderbos, Joost, et al. “Multiple Andreev reflections and Shapiro steps in a Ge-Si nanowire Josephson junction.” Physical Review Materials 3.8 (2019): 084803.